ABSTRACT
It has been well established that, in addition to the pulmonary involvement, COPD has systemic consequences that can lead to peripheral muscle dysfunction, with greater muscle fatigue, lower exercise tolerance and lower survival in these patients. In view of the negative repercussions of early muscle fatigue in COPD, the objective of this review was to discuss the principal findings in the literature on the metabolic and bioenergy determinants of muscle fatigue, its functional repercussions, as well as the methods for its identification and quantification. The anatomical and functional substrate of higher muscle fatigue in COPD appears to include lower levels of high-energy phosphates, lower mitochondrial density, early lactacidemia, higher serum ammonia and reduced muscle perfusion. These alterations can be revealed by contraction failure, decreased firing rates of motor units and increased recruitment of motor units in a given activity, which can be functionally detected by a reduction in muscle strength, power and endurance. This review article also shows that various types of muscle contraction regimens and protocols have been used in order to detect muscle fatigue in this population. With this understanding, rehabilitation strategies can be developed in order to improve the resistance to muscle fatigue in this population.
Keywords:
Pulmonary disease, chronic obstructive; Neuromuscular manifestations; Muscle fatigue; Exercise tolerance; Energy metabolism; Evaluation.
RESUMO
Está bem estabelecido que, além do acometimento pulmonar, a DPOC apresenta consequências sistêmicas que podem convergir para a disfunção muscular periférica, com maior fadigabilidade muscular, menor tolerância ao exercício e menor sobrevida nesses pacientes. Tendo em vista as repercussões negativas da fadiga muscular precoce na DPOC, esta revisão teve como objetivo discutir os principais achados da literatura relacionados aos seus determinantes metabólicos e bioenergéticos e suas repercussões funcionais, bem como seus métodos de identificação e de quantificação. O substrato anatomofuncional da maior fadigabilidade muscular na DPOC parece incluir a redução dos níveis de fosfatos de alta energia, a redução da densidade mitocondrial, a lactacidemia precoce, o aumento da amônia sérica e a perfusão muscular reduzida. Essas alterações podem ser evidenciadas por falência de contração, redução da taxa de disparo das unidades motoras e maior recrutamento de unidades motoras numa dada atividade, exteriorizando-se funcionalmente por redução da força, potência e resistência musculares. Esta revisão mostra também que diversos tipos de regimes contráteis e protocolos têm sido utilizados com o intuito de detectar fadiga nessa população. A partir de tais conhecimentos, estratégias reabilitadoras podem ser traçadas visando o aumento da resistência à fadiga muscular nessa população.
Palavras-chave:
Doença pulmonar obstrutiva crônica; Manifestações neuromusculares; Fadiga muscular; Tolerância ao exercício; Metabolismo energético; Avaliação.
IntroduçãoA intolerância ao exercício físico constitui um aspecto central na DPOC, estando associada à progressiva incapacidade e à redução da sobrevida. Os determinantes fisiopatológicos fundamentais são classicamente relacionados a um desequilíbrio crônico entre a elevada necessidade ventilatória e a reduzida capacidade de atendê-la.(1) Estudos têm mostrado que, além do acometimento pulmonar, a disfunção muscular periférica (DMP) contribui para a redução da capacidade de exercício(2) e da sobrevida desses pacientes.(3)
Os mecanismos relacionados à DMP na DPOC não estão totalmente elucidados; porém, a DMP tem sido caracterizada pela presença de atrofia, perda de força e de potência, além de fadiga muscular precoce, o que explica a intolerância ao exercício nesses pacientes.(2)
Classicamente, a fadiga muscular é conceituada como a incapacidade do músculo em gerar ou em manter níveis de força requeridos para uma determinada taxa de trabalho.(4) A fadiga muscular pode ser dividida em dois componentes: central e periférica. Essa terminologia se dá pelos sítios anatomofuncionais envolvidos no processo da fadiga. Na fadiga central, a ativação muscular a partir do sistema nervoso central está comprometida, enquanto, na fadiga periférica ou contrátil, o envolvimento é distal à junção neuromuscular.(5) Pode-se visualizar na Figura 1 a ilustração representativa das estruturas envolvidas na fadiga central e periférica.
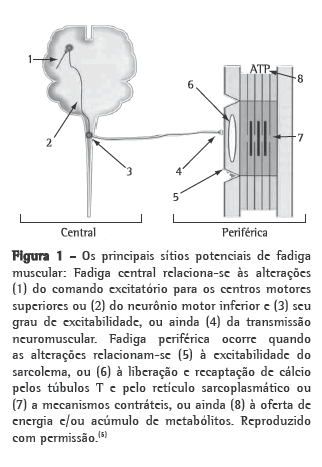
O interesse no estudo da fadiga muscular periférica em pacientes com DPOC é muito atual, pois o foco científico sempre se concentrou na contribuição da fadiga muscular respiratória e em suas implicações na tolerância ao exercício. Pode-se considerar que um estudo do início da década de 90 foi o precursor de subsequentes pesquisas sobre a fadiga muscular periférica, com uma contribuição quanto à limitação do exercício, pois o cansaço em membros inferiores foi o sintoma predominante ao interromper o exercício máximo em 43% da amostra de pacientes com DPOC do estudo. Para aquela época, os autores relataram que esse foi um resultado inesperado.(6) Nessa mesma década, outros autores demonstraram que a força muscular do quadríceps foi um importante determinante da capacidade física dessa população.(7) Esses achados tiveram um impacto significativo para o aprofundamento do estudo das relações entre desempenho muscular e capacidade de exercício. Mais recentemente, alguns autores demonstraram que a força do quadríceps é um previsor de mortalidade melhor do que a idade, índice de massa corpórea e VEF1 em pacientes com DPOC moderada a grave.(3)
Tendo em vista as repercussões negativas da fadiga muscular periférica na DPOC, esta revisão teve como objetivo discutir os principais achados da literatura relacionados aos seus determinantes metabólicos e bioenergéticos, bem como seus métodos de identificação e de quantificação. As bases de dados PubMed, Medline, SciELO e LILACS foram consultadas utilizando-se os seguintes descritores: doença pulmonar obstrutiva crônica, manifestações neuromusculares, fadiga muscular, tolerância ao exercício, metabolismo energético e métodos de avaliação.
Determinantes metabólicos e bioenergéticos da fadiga muscular periférica na DPOCComo abordado previamente, a DMP tem substancial contribuição na fadiga muscular periférica. O impacto dessa disfunção no desempenho muscular se desenvolve por anormalidades na estrutura,(8) na bioenergética(9) e na função muscular.(7) Algumas implicações clínicas importantes estão relacionadas às alterações da estrutura (massa) e função (força e resistência) muscular, como a redução da capacidade de exercício, da qualidade de vida e da sobrevida desses pacientes.(3,4,10)
A etiologia dessas anormalidades parece ser multifatorial, envolvendo fatores como descondicionamento, hipóxia e/ou hipercapnia, estresse oxidativo, senescência, disfunção hormonal, inflamação sistêmica, uso crônico ou repetitivo de fármacos (corticosteroides) e depleção nutricional.(2)
Fatores inflamatórios locais e/ou sistêmicos têm sido identificados na DPOC. Em pacientes estáveis, elevados níveis de proteína C reativa (PCR), fibrinogênio, leucócitos circulantes e citocinas pró-inflamatórias, incluindo TNF-α, IL-8 e IL-6, têm sido observados. Recentemente, elevações séricas da IL-18 também foram identificadas em pacientes com DPOC moderada a grave.(11-15)
O envolvimento dos mediadores inflamatórios na DMP é sugerido pela observação de que marcadores inflamatórios sistêmicos relacionam-se com baixo desempenho muscular contrátil na DPOC. Por exemplo, a força do quadríceps associou-se negativamente com os níveis de IL-8 durante a exacerbação da doença(15) e com os níveis de IL-6 e TNF-α em idosos.(16) Em pacientes muito graves, os níveis elevados de PCR associaram-se com a redução da força, da resistência e da qualidade de vida.(17)
Por outro lado, em outro estudo foi evidenciado que a fraqueza acentuada do quadríceps estava significantemente relacionada à média da dose diária de esteroides usada pelos pacientes durante a exacerbação aguda da doença. Os efeitos deletérios dos esteroides na função muscular esquelética têm sido atribuídos à inibição da síntese proteica e ao aumento da degradação proteica. Entretanto, devido ao fato de que os pacientes recebem corticosteroides para o tratamento da inflamação aguda, torna-se difícil a distinção entre o efeito muscular da administração dos esteroides e a ação deletéria direta da exacerbação da DPOC.(18)
Outras evidências sugerem a participação do estresse oxidativo na disfunção muscular de pacientes com DPOC.(15) Um desequilíbrio entre antioxidantes e oxidantes pode ser hipotetizado. Um grupo de autores estabeleceu uma relação inversa entre a magnitude do estresse oxidativo e a resistência do quadríceps em pacientes com DPOC.(19) Além disso, esse mesmo grupo mostrou que a terapia a curto prazo com altas doses de N-acetilcisteína (um antioxidante) previne o estresse oxidativo e melhora significantemente a resistência do quadríceps.(20)
Adicionalmente, tem sido mostrado que a inflamação sistêmica e a hipoxemia estão fortemente associados ao aumento do estresse oxidativo em pacientes com DPOC.(21) A hipoxemia crônica parece também ter envolvimento na redução da força e resistência musculares, aumentando a atrofia e atenuando a atividade enzimática mitocondrial. Sugere-se também que esses efeitos são rapidamente revertidos pela suplementação de oxigênio.(22)
Outros autores também mostraram que o ATP, os níveis de glicogênio e de phosphocreatine (PCr, fosfocreatina) estão significantemente reduzidos nas fibras musculares do quadríceps de pacientes com DPOC hipoxêmicos.(23)
A senescência parece ser um importante fator relacionado à perda de força muscular em pessoas saudáveis. Existe, aproximadamente, um declínio de 30% na força e de 40% na massa muscular entre a segunda e a sétima década de vida.(24) Esse declínio da força muscular está associado ao grau de atrofia das fibras do tipo II, e, geralmente, não são limitadas aos membros inferiores. Uma vez que, frequentemente, pacientes com DPOC apresentam idade avançada, os efeitos dessa doença na função muscular esquelética devem ser considerados.
Finalmente, o sedentarismo adotado para evitar ou minimizar a sensação de dispneia e de fadiga muscular promove o descondicionamento muscular, o qual resulta em fraqueza por redução da atividade do neurônio motor. Essa cascata culmina com a diminuição da proporção das fibras do tipo I, o aumento da proporção das fibras do tipo IIx e a redução do potencial oxidativo. No mesmo sentido, foi evidenciada significante correlação entre a inatividade física e a resistência do quadríceps em pacientes com DPOC.(25) Cabe salientar que essas alterações ocorrem mais pronunciadamente nos músculos locomotores desses pacientes.(2,10)
Uma recente revisão sistemática estabeleceu que, com base em valores de referência, é frequente uma redução de 27% na proporção de fibras do tipo I e um aumento de 29% na proporção de fibras do tipo IIx em pacientes com DPOC grave.(26)
O elevado gasto energético basal nas atividades de vida diária sugere um alto custo devido ao aumento da demanda ventilatória.(2) Além disso, a baixa ingestão proteico-calórica, causada pelos sintomas de dispneia, fadiga e saciedade precoce, faz com que ocorra um desequilíbrio entre a demanda e o consumo energético desses pacientes, contribuindo para o aumento do dispêndio energético diário dos mesmos.(27) A redução do índice de massa corpórea de pacientes com DPOC correlaciona-se com um pobre desempenho contrátil muscular, sugerindo que aspectos nutricionais possuem um papel determinante na função muscular desses pacientes.(28)
Alguns autores demonstraram que pacientes com DPOC e depleção muscular apresentaram maior queixa de fadiga de membros inferiores no teste de caminhada de seis minutos do que pacientes com DPOC e massa muscular preservada.(29)
Do ponto de vista microestrutural, as alterações histoquímicas e bioenergéticas observadas na musculatura esquelética podem ser resumidas da seguinte forma: manutenção ou elevação da atividade enzimática glicolítica; redução da capacidade oxidativa; diminuição da densidade capilar; redução da área de secção transversa das fibras lentas e rápidas; redução da atividade mitocondrial; e redução da relação capilar/fibra. Tais alterações aumentam a contribuição do sistema anaeróbico na geração de energia, resultando em lactacidemia precoce.(30)
Em síntese, a associação das anormalidades na estrutura, na bioenergética e na função dos músculos periféricos confere expressiva fadigabilidade muscular periférica nesses pacientes. Essa pode ainda ser intensificada nos quadros de exacerbação da doença.(7) Um resumo dos determinantes metabólicos e bioenergéticos da fadiga muscular em pacientes com DPOC pode ser visualizado no Quadro 1.
Métodos de determinação da fadiga muscular periférica na DPOCAtualmente, existe uma grande variedade de modelos, testes e protocolos de exercícios sendo utilizados, objetivando a obtenção de parâmetros indicativos da fadiga muscular em pacientes com DPOC.(4)
Os métodos de avaliação funcional de fadiga muscular periférica envolvem basicamente três componentes básicos:
i) a estratégia de ativação muscular: esforço voluntário ou estimulação exógena
ii) a condição do exercício (isométrico, isoinercial ou isocinético)
iii) o padrão de exercício: tempo e intensidade, podendo envolver uma única contração prolongada ou uma série de contrações repetidas
A maioria dos métodos utilizados nessa avaliação em pacientes com DPOC tem, preferencialmente, foco nos músculos dos membros inferiores. Cabe ressaltar, no entanto, que a função dos membros superiores mantém-se relativamente preservada nesses pacientes.
Contração voluntária máxima e submáximaA avaliação da fadiga muscular pela contração voluntária máxima (CVM) é obtida instruindo-se o paciente a gerar a maior força voluntária possível, sem alterar o comprimento muscular. Nessa avaliação, equipamentos como os tensiômetros ou dinamômetros são utilizados para a quantificação da força isométrica gerada. Por se tratar de uma técnica volitiva, complicadores externos, tais como a habilidade funcional e a motivação do paciente, podem gerar contrações com ativação submáxima.(31,32) Entretanto, devido à facilidade da execução, ao encorajamento verbal e à experiência técnica, pouca variabilidade tem sido descrita na CVM. Em pacientes com DPOC, a perda da capacidade de gerar ou manter a CVM durante o exercício de extensão do joelho tem sido representativo de fadiga muscular.(7,31,32)
Estudos têm utilizado séries de contrações voluntárias submáximas na avaliação da fadiga muscular de pacientes com DPOC.(25-28) Habitualmente, valores relativos de aproximadamente 20-60% da CVM são requeridos para serem sustentados até a exaustão. A resistência é medida pelo tempo no qual a tarefa é mantida. O teste deve ser interrompido quando há falência em manter a intensidade da contração (queda de 5%).(4,25,33)
Dinamometria isocinéticaO movimento isocinético consiste de contrações musculares em velocidade constante durante toda a amplitude do movimento. Esse tipo de contração só é obtido pelo uso de equipamentos isocinéticos, nos quais a resistência é variável e acomodativa, adaptando-se à força exercida. Com esses equipamentos, é possível obter a força desenvolvida em um ângulo de movimento e a duração da contração. Portanto, além da força, é possível a obtenção da variável trabalho, que é resultante do produto da força pela velocidade e potência, que é o trabalho realizado por unidade de tempo.
Quando se realiza a avaliação isocinética, pico de torque é o termo mais apropriado para expressar a força máxima gerada, pois a força ocorre em função do movimento rotacional da articulação em relação a um eixo.
Para a avaliação de força, são recomendadas velocidades angulares mais baixas, tipicamente 60-90°/s.(34) Para avaliação da resistência, as velocidades angulares mais utilizadas são 180°/s(30) e 300°/s.(7,34) Um maior torque é gerado quando se aplicam velocidades angulares baixas. Dessa forma, na avaliação de força, 3-5 repetições são utilizadas, sendo o mais alto valor obtido correspondente ao pico de torque. Na avaliação da resistência, utiliza-se 15-30 repetições sucessivas, pois menores picos de torque são gerados, sendo possível um maior número de contrações musculares.
Os resultados do teste de resistência podem ser interpretados de duas formas: trabalho total, que corresponde à soma da área sob a curva das contrações musculares; ou declínio no pico de torque ou trabalho ao longo de 15, 20, 30 ou 50 repetições.(30) O declínio é calculado dividindo-se o pico de torque ou o trabalho das cinco últimas contrações por aquele das cinco primeiras, e esse resultado é expresso em porcentagem.
Quanto maior o declínio, maior a fadiga desenvolvida.
Em populações clínicas, o mais recomendado é usar o trabalho total, uma vez que os pacientes já iniciam o teste gerando menor pico de torque em cada contração; sendo assim, sua redução não é pronunciada.
A vantagem mais óbvia de se usar a avaliação isocinética é o controle da velocidade e do ângulo do movimento, sendo sua confiabilidade e reprodutibilidade superiores às das técnicas convencionais.(35) Por outro lado, as desvantagens relacionam-se ao custo excessivo, à necessidade de treinamento do avaliador e à escassa validade externa, já que a velocidade angular constante não se constitui em um movimento fisiológico.(36)
Habitualmente, em pacientes com DPOC, o torque isométrico, o torque isocinético e o trabalho total se mostraram reduzidos quando comparados a controles saudáveis.(30,37)
Eletromiografia de superfícieA eletromiografia de superfície (EMGs) é uma ferramenta amplamente utilizada como método não-invasivo de avaliação da função muscular por meio da colocação de eletrodos sobre a pele. O estudo da função muscular pela EMGs resulta da análise da atividade elétrica do músculo por meio da determinação da somatória dos potenciais de ação de todas as fibras musculares.(38,39) Além da determinação da atividade elétrica muscular, a EMGs tem sido utilizada para a análise de fadiga, avaliação do treinamento, relação sinal EMGs/força e identificação de condições patológicas.(40,41)
Durante a contração muscular, o registro eletromiográfico basicamente proporciona dois parâmetros: raiz quadrada da média (RQM) e frequência mediana (FM). A RQM representa a amplitude do sinal eletromiográfico, ou seja, ela quantifica a atividade elétrica durante a contração. A FM está relacionada à taxa de disparo da unidade motora. Portanto, a fadiga muscular está presente quando, na análise eletromiográfica, observa-se(35):
aumento nos valores da RQM, que resulta da maior ativação muscular devido à redução na capacidade de sustentar a contração
redução nos valores de FM devido à redução no potencial de ação das fibras em contração muscular
O sinal eletromiográfico resultante desse método é um potencial de ação denominado onda M. A onda M é proporcional aos números de unidades motoras despolarizadas e, consequentemente, é um reflexo do tamanho da ativação das fibras musculares produzidas durante uma contração muscular. Uma redução da força contrátil com onda M inalterada é indicativa de fadiga contrátil, ou seja, falência do acoplamento excitação-contração.(42) Entretanto, quando a redução na força de contração está associada a uma diminuição da amplitude da onda M, pode-se suspeitar de falência de transmissão. Dessa forma, os possíveis sítios de disfunção muscular durante o exercício podem ser determinados.(4,35)
A EMGs vem sendo amplamente utilizada no âmbito da reabilitação pulmonar para avaliar a função muscular, inclusive durante o exercício.(43) Nesse contexto, autores compararam as alterações eletrofisiológicas do quadríceps femoral de pacientes com DPOC, levemente hipoxêmicos no exercício, e aquelas de indivíduos saudáveis durante o teste incremental em cicloergômetro através da EMGs.(44) Os pacientes com DPOC apresentaram menores valores de FM e maiores valores de RQM em relação ao grupo controle. Na sequência desse estudo, outros autores evidenciaram, num estudo randomizado, modificações das respostas eletromiográficas do quadríceps femoral durante o exercício realizado com a utilização de oxigênio. Os mesmos autores sugeriram que essas modificações estariam associadas à melhora do metabolismo aeróbio, ao retardo da fadiga, à maior excitabilidade e à maior ativação muscular num dado nível de exercício quando do uso de oxigênio.(45)
Em outro estudo, os pacientes com DPOC apresentaram menor capacidade de sustentar a contração isométrica sustentada do quadríceps femoral até a exaustão em 60% da contração voluntária em relação a indivíduos saudáveis (42 s e 80 s, respectivamente; p < 0,05). Apesar do menor tempo de resistência muscular, a queda na FM foi semelhante em ambos os grupos.(33)
TwitchTécnicas de avaliação não-volitivas da fadiga muscular têm a vantagem de assegurar a ativação máxima muscular, independentemente da colaboração do indivíduo. O twitch é um método indireto para a avaliação de fadiga, no qual a contração é elicitada por um único estímulo elétrico ou magnético, oferecido ao nervo.
Uma avaliação mais sensível de fadiga por esse método é a interpolação do twitch. Nessa técnica, solicita-se ao indivíduo realizar a CVM, e, adicionalmente, aplica-se o estímulo ao nervo; concomitantemente, o registro da atividade elétrica mostrará o recrutamento adicional de unidades motoras com o twitch. Quanto maior a diferença de atividade muscular gerada entre a CVM isolada e a CVM associada ao twitch, maior é a fadigabilidade muscular. Uma diferença > 15% entre a CVM e o twitch interpolado é caracterizado como fadiga contrátil. Essa técnica interpolada permite diferenciar a fadiga central da fadiga periférica; além disso, associada à onda M, é possível detectar a falência de transmissão ou de ativação central com esse método.(4)
O twitch vem sendo atualmente utilizado em âmbito científico para o entendimento dos efeitos do exercício dinâmico(32,46) e do exercício localizado na fadiga contrátil do quadríceps em pacientes com DPOC.(31,43,47,48) Entretanto, apesar de atualmente ser considerado o padrão ouro nessa avaliação, ainda não é um método disponível na prática clínica.(4)
Potência críticaUma abordagem fisiológica na avaliação da fadiga é o teste de capacidade ou de potência anaeróbia. Com esse método, procura-se avaliar a habilidade orgânica em prover energia sob condições independentes do aporte e/ou da utilização de oxigênio, ou seja, avalia-se a capacidade do indivíduo em sustentar um trabalho predominantemente anaeróbio. Nesse contexto, o conceito potência crítica (PC) envolve a realização de atividades de alta intensidade e de curta duração; por meio de um modelo matemático, determinam-se os limites de tolerância ao exercício. A importância clínica desse teste consiste em quantificar a carga de trabalho na qual a fadiga ocorre, ou seja, determinar o limiar de fadiga.(37) Embora envolva uma série de testes de exercícios dinâmicos extenuantes, esse protocolo foi factível em pacientes com DPOC.(37,49) Assim, alguns autores demonstraram que, nesses pacientes, a PC e a capacidade de trabalho anaeróbia apresentam determinantes diferentes de controles saudáveis, sendo essas dependentes da limitação ventilatória e da consequente sensação de dispneia nos pacientes com DPOC.(37)
Escalas de percepção de fadigaA avaliação da percepção subjetiva do sintoma de fadiga pelos pacientes durante os testes de exercício ou de treinamento tem sido comumente realizada por escalas. Embora possam ser encontradas mais de 30 escalas, as mais utilizadas são a escala modificada de Borg(50) e a escala analógica visual.(51) Embora de fácil aplicação, essas podem ter seus desfechos influenciados por diversos fatores, como motivação e compreensão. Portanto, embora amplamente utilizadas, essas devem ser associadas a outras avaliações objetivas.
Espectroscopia por ressonância magnética com fósforo 31A espectroscopia por ressonância magnética com fósforo 31 (ERM-P31) é um método de mensuração direta e não-invasiva da bioenergética muscular (ATP, PCr, fosfato inorgânico [PI] e pH intracelular) durante o exercício localizado repetitivo e recuperação posterior. Esse método tem sido clinicamente aplicado em pacientes com DPOC grave, com evidências de alterações pronunciadas no seu metabolismo muscular. Essas alterações se manifestam pela redução da capacidade aeróbica (↓PCr/Pi e ↓PCr/[PCr +PI]) durante o exercício, como também pelo menor pH intracelular e menor ressíntese de PCr durante a fase de recuperação do exercício quando comparadas às de indivíduos controle.(52-54)
Também foi mostrado que a administração aguda de oxigênio melhora parcialmente esses índices,(54) sugerindo que outros fatores além da hipoxemia afetam o metabolismo muscular.
Biópsia muscularEm um estudo utilizando técnicas de biópsia do músculo vasto lateral, foi demonstrada uma relação força/frequência elevada em pacientes com DPOC grave, ou seja, tais pacientes necessitaram de uma elevada frequência de estimulação para produzir a mesma força relativa do músculo vasto lateral quando comparados a controles normais.(55)
Adicionalmente, estudos com biópsia muscular nessa população mostraram atividade enzimática e metabólica alteradas, assim como alterações na proporção dos tipos de fibras musculares, bem como suas relações com a intolerância ao exercício.(8,9)
Dosagem de lactato e de amônia séricaMensurações de lactato no sangue durante o exercício dinâmico e no período de recuperação indicam que os pacientes com DPOC que interromperam o exercício por queixa de fadiga de membros inferiores tendem a apresentar níveis de lactato significantemente maiores do que os que interromperam por queixa de dispneia. Assim, alguns autores observaram, nos pacientes com fadiga, uma relação entre o aumento dos níveis de lactato e a queda no twitch do quadríceps após o exercício dinâmico.(56)
O início precoce da acidose lática observada nesses pacientes ocorre, principalmente, devido à reduzida densidade mitocondrial e à reduzida capacidade oxidativa muscular, levando à queda do pH e ao acúmulo de acido lático resultante da inabilidade da conversão do oxigênio em energia em situações ácidas.(43)
Outro marcador para monitorar a fadiga nesses pacientes é o nível de amônia sérica, a qual normalmente acompanha a resposta ao lactato durante o exercício. Em um estudo, foi observado que 60% dos pacientes com DPOC exibiram um aumento de amônia sérica durante o exercício, em comparação com indivíduos saudáveis. É provável que esse evento ocorra devido à degradação da enzima AMP desaminase, posteriormente convertida em monofosfato de inosina e amônia, com consequente redução na produção de ATP, tornando seu nível insuficiente para atender a demanda energética.(57)
Espectroscopia por raios quase infravermelhosAlterações fisiológicas, como perfusão muscular reduzida e hipoxemia, são achados comuns em pacientes com DPOC,(2) e ambas têm sido consideradas fatores potenciais para o desenvolvimento de fadiga contrátil. A espectroscopia por near infrared spectroscopy (NIRS, espectroscopia por raios quase infravermelhos) constitui uma tecnologia de ponta para a mensuração não-invasiva de indicadores da perfusão periférica, como a oxigenação muscular. Nessa técnica, as diferenças dependentes de oxigênio no espectro de absorção de ferro (no grupo prostético heme da hemoglobina) ou de cobre (em citocromo oxidase) tornam possível a estimativa das modificações na quantidade desses metais, fornecendo os volumes locais de oxi- e desoxi-hemoglobina, assim como a somatória de ambos os volumes no fluxo sanguíneo muscular local.(58,59)
Alguns autores mostraram, utilizando essa técnica em pacientes com DPOC, que o aumento do trabalho ventilatório no exercício moderado a intenso promove a redistribuição do fluxo sanguíneo, preferencialmente para os músculos respiratórios, reduzindo a perfusão muscular esquelética com consequente aumento da lactacidemia e da fadigabilidade muscular.(58) Recentemente, outros autores mostraram de forma mais eloquente, por meio de NIRS, uma maior extração na fração de oxigênio muscular periférico acompanhada de uma menor perfusão muscular estimada durante o exercício intenso de pacientes com DPOC quando comparada à do grupo controle.(59) Dessa forma, o evidente desequilíbrio entre a oferta e a utilização de oxigênio também desempenha um papel negativo na tolerância ao exercício desses pacientes.(58)
Diante das evidências do comprometimento da função muscular periférica e dos diferentes mecanismos que determinam a suscetibilidade à fadiga de pacientes com DPOC, o conhecimento dos distintos métodos de avaliação funcional disponíveis (Quadro 2) torna-se de fato necessário para que se possa discriminar o principal componente envolvido no processo de fadiga muscular periférica desses pacientes. Entretanto, deve-se também considerar alguns aspectos na escolha do método de avaliação, como a disponibilidade e a confiabilidade do equipamento, sua relação custo-benefício, além da facilidade operacional e da colaboração do paciente.
Considerações finaisO substrato anatomofuncional da maior fadigabilidade muscular na DPOC parece incluir a redução dos níveis de fosfatos de alta energia, a densidade mitocondrial, a lactacidemia precoce, o aumento da amônia sérica e a perfusão muscular reduzida. Essas alterações podem ser evidenciadas por falência de contração, redução da taxa de disparo da unidade motora e maior recrutamento de unidades motoras numa dada atividade, exteriorizando-se funcionalmente por redução da força, potência e resistência musculares. A presente revisão mostra também que diversos tipos de regimes contráteis e protocolos têm sido utilizados com o intuito de detectar fadiga em pacientes com DPOC. A partir de tais conhecimentos, estratégias reabilitadoras podem ser traçadas visando o aumento da resistência à fadiga muscular nessa população.
Referências 1. Global Initiative for Chronic Obstructive Lung Disease. Global strategy for the diagnosis, management, and prevention of chronic obstructive pulmonary disease. Bethesda: Global Initiative for Chronic Obstructive Lung Disease; 2007.
2. Skeletal muscle dysfunction in chronic obstructive pulmonary disease. A statement of the American Thoracic Society and European Respiratory Society. Am J Respir Crit Care Med. 1999;159(4 Pt 2):S1-40.
3. Swallow EB, Reyes D, Hopkinson NS, Man WD, Porcher R, Cetti EJ, et al. Quadriceps strength predicts mortality in patients with moderate to severe chronic obstructive pulmonary disease. Thorax. 2007;62(2):115-20.
4. Vøllestad NK. Measurement of human muscle fatigue. J Neurosci Methods. 1997;74(2):219-27.
5. Neder JA, Neder LE. Fisiologia Clínica do Exercício: Teoria e Prática. São Paulo: Artes Médicas; 2003.
6. Killian KJ, Leblanc P, Martin DH, Summers E, Jones NL, Campbell EJ. Exercise capacity and ventilatory, circulatory, and symptom limitation in patients with chronic airflow limitation. Am Rev Respir Dis. 1992;146(4):935-40.
7. Gosselink R, Troosters T, Decramer M. Peripheral muscle weakness contributes to exercise limitation in COPD. Am J Respir Crit Care Med. 1996;153(3):976-80.
8. Whittom F, Jobin J, Simard PM, Leblanc P, Simard C, Bernard S, et al. Histochemical and morphological characteristics of the vastus lateralis muscle in patients with chronic obstructive pulmonary disease. Med Sci Sports Exerc. 1998;30(10):1467-74.
9. Maltais F, Simard AA, Simard C, Jobin J, Desgagnés P, LeBlanc P. Oxidative capacity of the skeletal muscle and lactic acid kinetics during exercise in normal subjects and in patients with COPD. Am J Respir Crit Care Med. 1996;153(1):288-93.
10. Dourado VZ, Tanni SE, Vale SA, Faganello MM, Sanchez FF, Godoy I. Systemic manifestations in chronic obstructive pulmonary disease. J Bras Pneumol. 2006;32(2):161-71.
11. Schols AM, Buurman WA, Staal van den Brekel AJ, Dentener MA, Wouters EF. Evidence for a relation between metabolic derangements and increased levels of inflammatory mediators in a subgroup of patients with chronic obstructive pulmonary disease. Thorax. 1996;51(8):819-24.
12. Dentener MA, Creutzberg EC, Schols AM, Mantovani A, van't Veer C, Buurman WA, et al. Systemic anti-inflammatory mediators in COPD: increase in soluble interleukin 1 receptor II during treatment of exacerbations. Thorax. 2001;56(9):721-6.
13. Gan WQ, Man SF, Senthilselvan A, Sin DD. Association between chronic obstructive pulmonary disease and systemic inflammation: a systematic review and a meta-analysis. Thorax. 2004;59(7):574-80.
14. Elevated levels of IL-18 in plasma and skeletal muscle in chronic obstructive pulmonary disease. Lung. 2007;185(3):161-71.
15. Spruit MA, Gosselink R, Troosters T, Kasran A, Gayan-Ramirez G, Bogaerts P, et al. Muscle force during an acute exacerbation in hospitalised patients with COPD and its relationship with CXCL8 and IGF-I. Thorax. 2003;58(9):752-6.
16. Yende S, Waterer GW, Tolley EA, Newman AB, Bauer DC, Taaffe DR, et al. Inflammatory markers are associated with ventilatory limitation and muscle dysfunction in obstructive lung disease in well functioning elderly subjects. Thorax. 2006;61(1):10-6.
17. Broekhuizen R, Wouters EF, Creutzberg EC, Schols AM. Raised CRP levels mark metabolic and functional impairment in advanced COPD. Thorax. 2006;61(1):17-22.
18. Decramer M, Lacquet LM, Fagard R, Rogiers P. Corticosteroids contribute to muscle weakness in chronic airflow obstruction. Am J Respir Crit Care Med. 1994;150(1):11-6.
19. Richardson RS, Leek BT, Gavin TP, Haseler LJ, Mudaliar SR, Henry R, et al. Reduced mechanical efficiency in chronic obstructive pulmonary disease but normal peak VO2 with small muscle mass exercise. Am J Respir Crit Care Med. 2004;169(1):89-96.
20. Koechlin C, Couillard A, Cristol JP, Chanez P, Hayot M, Le Gallais D, et al. Does systemic inflammation trigger local exercise-induced oxidative stress in COPD? Eur Respir J. 2004;23(4):538-44.
21. Boots AW, Haenen GR, Bast A. Oxidant metabolism in chronic obstructive pulmonary disease. Eur Respir J Suppl. 2003;46:14s-27s.
22. Faucher M, Steinberg JG, Barbier D, Hug F, Jammes Y. Influence of chronic hypoxemia on peripheral muscle function and oxidative stress in humans. Clin Physiol Funct Imaging. 2004;24(2):75-84.
23. Jakobsson P, Jorfeldt L, Brundin A. Skeletal muscle metabolites and fibre types in patients with advanced chronic obstructive pulmonary disease (COPD), with and without chronic respiratory failure. Eur Respir J. 1990;3(2):192-6.
24. Lexell J. Human aging, muscle mass, and fiber type composition. J Gerontol A Biol Sci Med Sci. 1995;50 Spec No:11-6.
25. Serres I, Gautier V, Varray A, Préfaut C. Impaired skeletal muscle endurance related to physical inactivity and altered lung function in COPD patients. Chest. 1998;113(4):900-5.
26. Gosker HR, Zeegers MP, Wouters EF, Schols AM. Muscle fibre type shifting in the vastus lateralis of patients with COPD is associated with disease severity: a systematic review and meta-analysis. Thorax. 2007;62(11):944-9.
27. Schols AM, Soeters PB, Dingemans AM, Mostert R, Frantzen PJ, Wouters EF. Prevalence and characteristics of nutritional depletion in patients with stable COPD eligible for pulmonary rehabilitation. Am Rev Respir Dis. 1993;147(5):1151-6.
28. Kim HC, Mofarrahi M, Hussain SN. Skeletal muscle dysfunction in patients with chronic obstructive pulmonary disease. Int J Chron Obstruct Pulmon Dis. 2008;3(4):637-58.
29. Pelegrino NR, Lucheta PA, Sanchez FF, Faganello MM, Ferrari R, Godoy I. Influence of lean body mass on cardiopulmonary repercussions during the six-minute walk test in patients with COPD. J Bras Pneumol. 2009;35(1):20-6.
30. Malaguti C, Nery LE, Dal Corso S, Nápolis L, De Fuccio MB, Castro M, et al. Scaling skeletal muscle function to mass in patients with moderate-to-severe COPD. Eur J Appl Physiol. 2006;98(5):482-8.
31. Allen GM, Gandevia SC, McKenzie DK. Reliability of measurements of muscle strength and voluntary activation using twitch interpolation. Muscle Nerve. 1995;18(6):593-600.
32. Man WD, Soliman MG, Gearing J, Radford SG, Rafferty GF, Gray BJ, et al. Symptoms and quadriceps fatigability after walking and cycling in chronic obstructive pulmonary disease. Am J Respir Crit Care Med. 2003;168(5):562-7.
33. Allaire J, Maltais F, Doyon JF, Noël M, LeBlanc P, Carrier G, et al. Peripheral muscle endurance and the oxidative profile of the quadriceps in patients with COPD. Thorax. 2004;59(8):673-8.
34. Gleeson NP, Mercer TH. The utility of isokinetic knee extension and flexion strength characteristics of adult males: a comparative examination of gravity corrected and uncorrected data. J Sports Sci. 1996;14(5):415-6.
35. Man WD, Soliman MG, Nikoletou D, Harris ML, Rafferty GF, Mustfa N, et al. Non-volitional assessment of skeletal muscle strength in patients with chronic obstructive pulmonary disease. Thorax. 2003;58(8):665-9.
36. Mador MJ, Kufel TJ, Pineda LA, Steinwald A, Aggarwal A, Upadhyay AM, et al. Effect of pulmonary rehabilitation on quadriceps fatiguability during exercise. Am J Respir Crit Care Med. 2001;163(4):930-5.
37. Neder JA, Jones PW, Nery LE, Whipp BJ. Determinants of the exercise endurance capacity in patients with chronic obstructive pulmonary disease. The power-duration relationship. Am J Respir Crit Care Med. 2000;162(2 Pt 1):497-504.
38. De Luca CJ. The use of surface electromyography in biomechanics. J Appl Biomech. 1997;13(1):135-63.
39. Duchêne J, Goubel F. Surface electromyogram during voluntary contraction: processing tools and relation to physiological events. Crit Rev Biomed Eng. 1993;21(4):313-97.
40. Hausswirth C, Brisswalter J, Vallier JM, Smith D, Lepers R. Evolution of electromyographic signal, running economy, and perceived exertion during different prolonged exercises. Int J Sports Med. 2000;21(6):429-36.
41. Soderberg GL, Knutson LM. A guide for use and interpretation of kinesiologic electromyographic data. Phys Ther. 2000;80(5):485-98.
42. Farina D, Blanchietti A, Pozzo M, Merletti R. M-wave properties during progressive motor unit activation by transcutaneous stimulation. J Appl Physiol. 2004;97(2):545-55.
43. Saey D, Côté CH, Mador MJ, Laviolette L, LeBlanc P, Jobin J, et al. Assessment of muscle fatigue during exercise in chronic obstructive pulmonary disease. Muscle Nerve. 2006;34(1):62-71.
44. Gosselin N, Matecki S, Poulain M, Ramonatxo M, Ceugniet F, Préfaut C, et al. Electrophysiologic changes during exercise testing in patients with chronic obstructive pulmonary disease. Muscle Nerve. 2003;27(2):170-9.
45. Gosselin N, Durand F, Poulain M, Lambert K, Ceugniet F, Préfaut C, et al. Effect of acute hyperoxia during exercise on quadriceps electrical activity in active COPD patients. Acta Physiol Scand. 2004;181(3):333-43.
46. Man WD, Soliman MG, Gearing J, Radford SG, Rafferty GF, Gray BJ, et al. Symptoms and quadriceps fatigability after walking and cycling in chronic obstructive pulmonary disease. Am J Respir Crit Care Med. 2003;168(5):562-7.
47. Verin E, Ross E, Demoule A, Hopkinson N, Nickol A, Fauroux B, et al. Effects of exhaustive incremental treadmill exercise on diaphragm and quadriceps motor potentials evoked by transcranial magnetic stimulation. J Appl Physiol. 2004;96(1):253-9.
48. Swallow EB, Gosker HR, Ward KA, Moore AJ, Dayer MJ, Hopkinson NS, et al. A novel technique for nonvolitional assessment of quadriceps muscle endurance in humans. J Appl Physiol. 2007;103(3):739-46.
49. Malaguti C, Nery LE, Dal Corso S, De Fuccio MB, Lerario MC, Cendon S, et al. Alternative strategies for exercise critical power estimation in patients with COPD. Eur J Appl Physiol. 2006;96(1):59-65.
50. Borg GA. Psychophysical bases of perceived exertion. Med Sci Sports Exerc. 1982;14(5):377-81.
51. Hayes M, Patterson D. Experiental development of the graphic rating method. Psychol Bull 1921;18:98-9.
52. Kutsuzawa T, Shioya S, Kurita D, Haida M, Ohta Y, Yamabayashi H. Muscle energy metabolism and nutritional status in patients with chronic obstructive pulmonary disease. A 31P magnetic resonance study. Am J Respir Crit Care Med. 1995;152(2):647-52.
53. Tada H, Kato H, Misawa T, Sasaki F, Hayashi S, Takahashi H, et al. 31P-nuclear magnetic resonance evidence of abnormal skeletal muscle metabolism in patients with chronic lung disease and congestive heart failure. Eur Respir J. 1992;5(2):163-9.
54. Lévy P, Wuyam B, Pépin JL, Reutenauer H, Payen JF. Skeletal muscle abnormalities in chronic obstructive lung disease with respiratory insufficiency. Value of P31 magnetic resonance spectroscopy [Article in French]. Rev Mal Respir. 1997;14(3):183-91. Erratum in: Rev Mal Respir. 1997;14(4):333.
55. Debigaré R, Côte CH, Hould FS, LeBlanc P, Maltais F. In vitro and in vivo contractile properties of the vastus lateralis muscle in males with COPD. Eur Respir J. 2003;21(2):273-8.
56. Saey D, Michaud A, Couillard A, Côté CH, Mador MJ, LeBlanc P, et al. Contractile fatigue, muscle morphometry, and blood lactate in chronic obstructive pulmonary disease. Am J Respir Crit Care Med. 2005;171(10):1109-15.
57. Calvert LD, Singh SJ, Greenhaff PL, Morgan MD, Steiner MC. The plasma ammonia response to cycle exercise in COPD. Eur Respir J. 2008;31(4):751-8.
58. Borghi-Silva A, Oliveira CC, Carrascosa C, Maia J, Berton DC, Queiroga F Jr, et al. Respiratory muscle unloading improves leg muscle oxygenation during exercise in patients with COPD. Thorax. 2008;63(10):910-5.
59. Chiappa GR, Borghi-Silva A, Ferreira LF, Carrascosa C, Oliveira CC, Maia J, et al. Kinetics of muscle deoxygenation are accelerated at the onset of heavy-intensity exercise in patients with COPD: relationship to central cardiovascular dynamics. J Appl Physiol. 2008;104(5):1341-50.
Trabalho realizado na Universidade Nove de Julho, São Paulo (SP) Brasil.
Endereço para correspondência: Carla Malaguti. Mestrado em Ciências da Reabilitação, Avenida Francisco Matarazzo, 612, Água Branca, CEP 05001-100, São Paulo, SP, Brasil.
Tel 55 11 3665-9748. Email: carlamalaguti@uninove.br
Apoio financeiro: Este estudo recebeu apoio financeiro da Universidade Nove de Julho, da Fundação de Amparo à Pesquisa do Estado de São Paulo (FAPESP) e do Conselho Nacional de Desenvolvimento Científico e Tecnológico (CNPq).
Recebido para publicação em 17/2/2009. Aprovado, após revisão, em 7/7/2009.
Sobre os autoresRafaella Rezende Rondelli
Pesquisadora Colaboradora. Programa de Mestrado em Ciências da Reabilitação, Universidade Nove de Julho, São Paulo (SP) Brasil.
Simone Dal Corso
Docente. Programa de Mestrado em Ciências da Reabilitação, Universidade Nove de Julho, São Paulo (SP) Brasil.
Alexandre Simões
Mestrando. Programa de Mestrado em Ciências da Reabilitação, Universidade Nove de Julho, São Paulo (SP) Brasil.
Carla Malaguti
Docente. Programa de Mestrado em Ciências da Reabilitação, Universidade Nove de Julho, São Paulo (SP) Brasil.